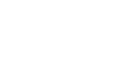
Protecting BESSs with comprehensive circuit strategy
Next-level power density in solar and energy storage with silicon carbide MOSFETs
Realizing the potential of CoolSiC™ MOSFETs for cost-effective power density in solar power generation and energy storage systems.
Introduction
The drive towards alternative, renewable energy sources is accelerating, with commitments from the major economies to move towards carbon neutrality in the relatively near future. Even the biggest emitters from east to west are now setting aggressive target dates. For example, the new administration in the US is looking to make electricity production carbon-free by 2035 with net zero emissions by 2050, and China is targeting a cut in ‘CO2 intensity of GDP’ by more than 65% from 2005 levels by 2030 [1]. This trend can be seen around the world, with some major countries such as Sweden already reaching more than 30% renewable electricity supply as of late 2020.
Of course, there is a range of renewable energy options, from geothermal to wind, hydro, biogas, tidal and solar. All have their challenges, whether in capital costs in hydro and wind, or pollutant emissions in biogas, or continuity of supply with wind and solar. However, photovoltaic (PV) arrays are attractive for their decreasing capital cost and ease of scalability from domestic to utility installations. If the continuity problem can be resolved with an energy storage system, then solar is a strong contender for future energy supply. Even though solar will always share the renewable energy market with other sources, growth in the industry has been strong and is predicted to increase exponentially (Figure 1).
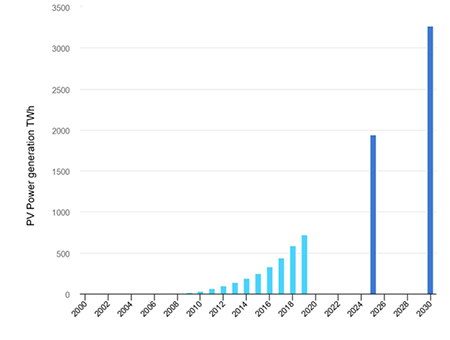
Figure 1: Solar PV power generation in the Sustainable Development Scenario, 2000-2030, source IEA, Paris [2]
Solar power generation structures
PV installations fall into three distinct categories: residential installations with up to 10 kW power, commercial installations reaching around 5 MW power, and utility installations at higher power still. Residential installations are typically seen as a long-term investment, in addition to the existing utility grid connection. They will often have local battery storage for excess solar energy, which provides ‘peak shaving’ and a useful back-up if the main AC supply fails during hours of darkness. Single or multiple PV panels, at typically 40 V to 80 V voltage, the microinverter and the battery installation can integrate as a system with increasing levels of web-connected home automation, to optimise energy use through scheduling of demand and storage. Another option is the integration of an electric car-charging system in the local network, with optional charging from solar energy or the usual AC supply grid. With bidirectional power conversion, the electric vehicle (EV) battery can form another energy storage element for domestic use or even to feed back into the utility supply for cash credit. A typical installation might look like the one shown in Figure 2.
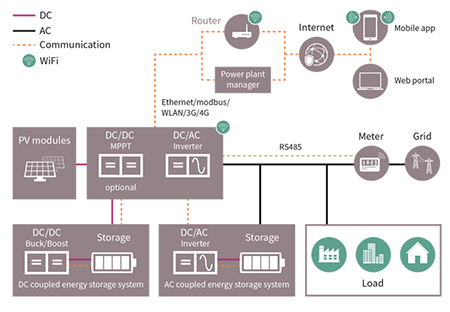
Figure 2: An example residential solar power installation with battery storage, EV integration and utility energy feed-in
Commercial solar installations, as an additional or even primary energy source for offices and factories, have similar requirements as residential installations. However, commercially, the higher energy demand implies higher equipment costs, so system efficiency and quick payback are key considerations. PV arrays will be in strings and voltages will be higher, perhaps up to 1500 V, to reduce current and consequential ohmic losses. Local battery energy storage will often be integrated to reduce peak utility demand, which attracts premium rates. One inverter will typically be allocated to one or a few PV strings in a bigger system for fault tolerance, scalability and convenience.
Large commercial PV and utility installations can use a single, central, three-phase inverter. The central approach is used mainly for remote large-scale installations above about 10 MW, where high power can be efficiently transformed and fed directly into a transmission grid. Below 10 MW, the disadvantages of a central inverter compared with string inverters are inflexibility, higher initial capital costs and lack of incremental scalability. A central inverter also risks supply continuity, as it is a single point of failure, so there is a trend towards distributed inverter systems with associated energy storage. Ultimately, the choice between a distributed string or central inverter arrangement is a complex decision, based on operation and maintenance costs, plant layout and design flexibility, ease of installation and access, power redundancy and much more.
A string inverter in a cabinet size with a weight of around 80 kg is seen as optimal, because it can be handled and installed or replaced by a two-person team. With this in mind, there is an intense effort to maximize the power rating of this size of cabinet by improving efficiency, and therefore, power density, without adding weight and cost. New power conversion topologies and semiconductor switch technologies are enablers for this.
PV inverter topologies – micro, string and central
Microinverters used for residential installations often integrate closely with the PV panel hardware and achieve moderate efficiency levels of around 96%. A microinverter may operate with a single low-voltage (<60 V) PV panel with an isolated DC-DC converter stage boosting to a high-voltage, regulated DC link, feeding a grid-compatible single-phase inverter. When multiple panels at higher power are used, it becomes necessary to use a more complex bridge circuit that combines and converts the panel voltages to a single DC-link output, feeding the inverter. Designs are targeted at low cost for a potential mass market, with switching frequencies typically in the 40 kHz–80 kHz range. The output is invariably single phase 110 V/230 VAC, and silicon MOSFET semiconductor switches are common, with IGBTs used in more basic installations if frequency is kept low. Because of the relatively low power of microinverters, the conversion topology is typically a flyback or perhaps a ‘LLC’ DC-DC stage with maximum power point tracking (MPPT) functionality, followed by a traditional bridge inverter for AC output. A ‘cycloinverter’ technique can also be used for single-stage conversion from PV DC to line AC.
In all configurations, the microinverter typically includes four to eight low-voltage switches and four highvoltage types. Energy storage can be provided by charging a battery from the inverter AC output using a bidirectional AC-DC converter allowing the battery to effectively replace the inverter output in low light conditions. The battery may also be charged from utility AC power as desired, with more complex systems allowing stored energy to be fed back into the AC line. In this way, the battery or energy storage system (ESS) can be programmed to charge from solar or utility AC when rates are low, and revert to backing up and storing solar energy when utility rates are higher.
String inverters used in residential, commercial and utility-scale installations will generate single- or alternatively three-phase AC power at higher levels. Panel voltages may be 600 V followed by a DC-DC boost converter to provide a DC link for a single-phase inverter. Alternatively, a 1000 VDC/1500 VDC system with a boost converter in a ‘3-level’ configuration is possible for a three-phase inverter DC link.
For single-phase AC, the inverter may be a simple 2-level implementation, or one of the topologies designed for improved efficiency such as the ‘HERIC’, ‘H6’ or multilevel types. The semiconductor switch count goes up with the topology complexity, but multilevel converters do allow use of lower voltagerated, and sometimes lower cost devices, albeit at the expense of more complex, multiple gate drives. As with microinverters, energy storage can be provided by batteries charged through a DC-DC converter off the PV panels. Because of the higher panel voltage, an isolated bidirectional converter would be used for safety reasons for less than 48 V battery. Battery charging from, or feed-in to, the utility AC supply can be implemented as needed.
Three-phase string inverters also vary in complexity depending on the power level, with simple 2-level, three-leg bridges used up to around 10 kW system power rating, and 3-level NPC1/NPC2/ANPC types operating into the 250-kW region. Complexity again scales with power, with the 3-level active neutral point clamped (ANPC) arrangement, for example, requiring a minimum of 18 high-voltage switches. In practice, integrated modules of multiple MOSFETs or IGBTs are typically used at the higher power levels.
Central inverters in utility-scale applications generate three-phase AC output at megawatt levels with the highest PV panel voltages and multilevel or paralleled inverters using typically IGBT modules. If local energy storage is provided, strings of batteries up to around 1000 V may be used with comprehensive battery management to ensure cell balancing and optimum service life. Feeding into the utility AC lines from the batteries provides load levelling or ‘peak shaving’ for the power network, independent of the solar energy generation. Figure 3 summarizes the application requirements across micro-, string and central inverters.
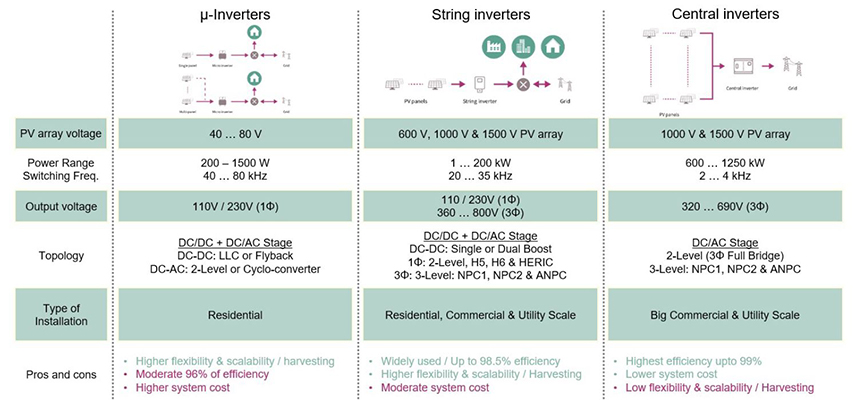
Figure 3: Application requirements for solar inverter categories
SiC switch technology
In all solar power applications, from residential to utility scale, efficiency of energy conversion is a key parameter. Every watt dissipated in equipment represents a step away from the goal of carbon neutrality and a reduction in the cost-effectiveness of the installation. Conversely, even a fraction of a percentage point saved can mean lower operating costs, smaller, lighter and cooler-running equipment, longer backup run time from batteries, and quicker capital payback.
Semiconductor switches employed in PV power conversion not only represent a significant loss contributor in themselves, but can also limit the choice of other components of the system. IGBTs for example, although they can have low static losses, cannot operate at very high frequency due to their slow switching, causing excessive dynamic losses. However, low-frequency operation generally requires larger and heavier magnetic and capacitive components. An ideal choice therefore is a switch that matches the on-state losses of an IGBT at high currents, but that can switch at higher frequency with fast edge rates. This will enable low dynamic loss and smaller passive components. Silicon MOSFETs are a contender at low power from a few kW to 10 kW, but lose out to IGBTs for static losses at high power, due to the MOSFET’s finite on-resistance. This is caused by the increase of power dissipated in a MOSFET channel resistance with the square of the current, whereas an IGBT has a near constant saturation voltage, with dissipation consequently just proportional to current.
A better proposition is a MOSFET using silicon carbide (SiC) technology, now ten years on from the launch of the first 1200 V device. SiC MOSFETs are wide band-gap semiconductors that have several advantages over silicon: critical breakdown field strength, so that the active layer is thinner at a given voltage rating and can be doped at higher level. Thus, the on-resistance is much lower for a given chip size. The smaller die dimensions also yield lower device capacitances, which allow faster switching with lower loss. Electron saturation velocity of SiC is anyway around twice the silicon value, enabling higher switching speed. Additionally, thermal conductivity of SiC is about three times better than silicon, allowing lower die temperatures for a given power dissipation and consequent lower uplift in on resistance.
Implementing SiC in solar technology
SiC MOSFETs up to 1200 V rating can be used directly in the MPPT DC-DC boost stage at up to 1000 V PV array voltage in residential, small and medium-scale commercial installations, and in the downstream single-phase or three-phase DC-AC inverter. In large commercial/utility installation panels with up to 1500 VDC output, SiC MOSFETs can still be used in a DC-DC 3-level boost arrangement, keeping the MOSFET voltage stress below the 1200 V rating. Subsequent three-phase inverters can be multilevel types, where the voltage is shared across series switches, again allowing 1200 V SiC MOSFETs to be used. DC-AC inverter switching frequency is not usually pushed very high; even though SiC can switch at MHz rates efficiently, inverters only have magnetic components for filtering rather than energy storage and coupling, so magnetics do not scale down as dramatically as in AC-DC or DC-DC converters, with their large transformers and storage chokes. SiC inverters switching around 100 kHz are therefore a good choice for very low dynamic and static loss, along with reasonable size filter components.
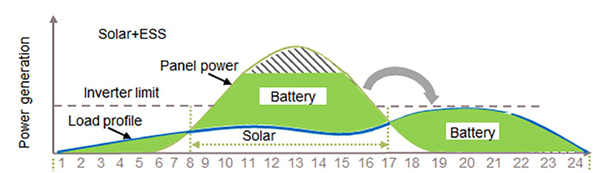
Figure 4: Over a day, solar and battery energy can fulfil load requirements
Installations with energy storage employ bidirectional DC-DC buck-boost converters for battery charging, and discharge to the local load with the battery being wall-mounted, in an EV, or both. Useable hours of a PV installation can be extended by controlling the contributions of solar and battery energy (Figure 4). A bidirectional, AC-DC/DC-AC power factor-corrected converter similarly provides battery energy to charge from, or feed into, the utility supply. These power conversion stages require ‘third quadrant’ or reverse switch conduction. In this mode, current momentarily flows through the MOSFET body diode before the device channel conducts, and the recovery of the stored charge leads to dynamic power loss when the channel turns off. SiC MOSFET body diodes have much lower stored charge (QRR) than silicon types, therefore improving conversion efficiency significantly. SiC MOSFET output capacitance and channel on-resistance is also comparatively lower than for silicon types, leading to yet more efficiency savings.
As an additional advantage, both uni- and bidirectional battery chargers boost DC-DC converters to provide DC links, while other associated power converters and power factor correction stages can all operate at higher frequency with SiC. This has the effect of reducing associated magnetic component size, weight, loss and cost dramatically. SiC MOSFETs can therefore be used to advantage in all power conversion stages in solar applications, yielding low overall losses and smaller passive components, with consequential lower energy and system costs, and longer back-up storage run-time.
Solution offering for solar and ESS
SiC MOSFETs are available from Infineon, under their CoolSiC™ brand, in 650 V, 1200 V and 1700 V classes. As discrete devices, the range includes parts with on-resistance as low as 27 milliohms and with drain current rating up to 56 amps. Discrete packages are surface-mount TO-263 and through-hole are TO-247, both available with Kelvin source connections for optimized switching performance. For multilevel converters, CoolSiC™ modules rated at 1200 V are also offered in 3-level, dual, four-pack and sixpack configurations, with on-resistance down to 2 milliohms. ‘Booster’ modules include dual SiC MOSFETs and SiC Schottky diodes, along with bypass diodes, to form a two-phase boost power stage, particularly suitable for PV voltage conversion to inverter DC-link input level. Power levels exceeding 200 kW for a single bidirectional AC-DC/DC-AC converter in a 1500 VDC PV system can be achieved at an efficiency close to 99% in both directions, ideally suitable for a high efficiency string or energy storage inverter.
As with all switches, SiC MOSFETs perform best when their gate drive is accurately controlled. Gate drive ICs from Infineon in their EiceDRIVER™ family can provide isolated high-side and low-side signals with lowest propagation delays, active Miller clamps and the ability to adjust or minimize deadtime – a vital feature to optimise a SiC MOSFET’s switching losses. Protection is also comprehensive with desaturation and short-circuit detection, along with common-mode transient immunity higher than 200 kV/µs across the isolation barrier, which also has pending 5.7 kVrms (1 min) safety rating. Isolation suits 1200 V-rated switches for all common DC-link and single/three-phase converter and inverter applications.For a complete ESS system solution, Infineon also provides components necessary for battery monitoring, protection and charge/discharge control. In energy storage systems, under-/overvoltage, inrush current, short-circuit and reverse currents can be detected and controlled while state of charge, state of health, temperature and cell balancing can be remotely indicated and controlled for optimum system lifetime and availability, all with high security through encryption and authentication processes (Figure 5).
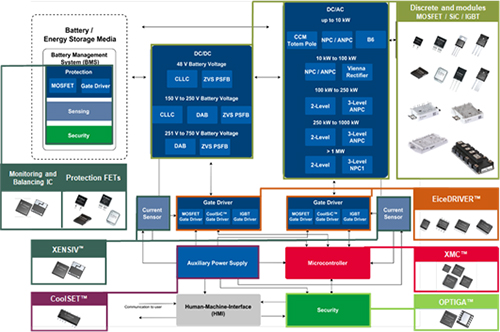
Figure 5: Infineon components provide a complete solution in energy storage and conversion systems
Reference designs prove the performance
A demonstration of the overall benefits of using SiC MOSFETs in PV string and energy storage inverters can show not only incremental savings in switch efficiency, but also wider advantages. Infineon has done this with a modular reference design for 1500 VDC systems rated up to 300 kW. The design uses a novel bidirectional 3-level ANPC topology which achieves better than 99.0% efficiency in both directions switching at up to 96 kHz. Power density is greater than 5 kW/kg for a complete solution including heatsinking and all control, allowing 300 kW throughput in the ideal 80 kg maximum cabinet weight. Energy usage and cost savings using SiC can be easily calculated from overall efficiency improvements. For example, compared with a super-junction Si MOSFET solution, 1200 V Cool SiCTM MOSFETs can lead to a halving of losses in an ESS installation, providing typically 2% extra energy and run-time. Compared with an IGBT inverter solution, comparably sized SiC MOSFET modules can also handle more power. For example, an Infineon 950 V EasyPACKTM 3B IGBT module operating at 16 kHz can be replaced by two smaller, 1200 V CoolSiC™ 2B IGBT modules at 32 kHz, with a 27% increase in power handling to 156 kVA.Although the SiC MOSFET unit cost is generally not yet lower than that of an IGBT for similar headline ratings, system hardware costs are lower due to maintained high efficiency at higher switching frequencies. This allows smaller and cheaper magnetic components and heat sinks to be implemented. For example, in a 1500 V PV string inverter, around 5% to 10% cost savings per kW can be expected (Figure 6).
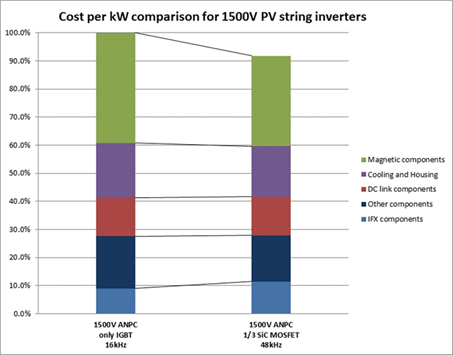
Figure 6: System costs in string inverters are significantly lower with SiC MOSFETs compared with IGBTs
SiC MOSFETs in solar and energy storage applications have clear benefits over other technologies, addressing the pressing need for energy and cost savings, particularly when bidirectional power conversion is required. Infineon offers a comprehensive range of SiC MOSFET discrete parts and modules, along with supporting drive, control and monitoring ICs for a complete system solution.
To learn more about Infineon CoolSiC™ solutions please click here.
Download this article in pdf