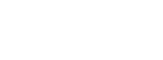
Current market and technological developments in automotive traction inverter systems
Introduction
The trend towards automotive electrification has accelerated worldwide in recent times, driven by the need for carbon neutrality and supported by stricter environmental regulations, core technology advancements, and declining costs. Most world regions are striving to tighten environmental regulations, with Europe leading the way, while greenhouse gas standards in other regions are following closely. Stricter GHG emission standards are on the way, which may lead to a temporary revitalization of the hybrid electric vehicle market as an early solution in the transition phase towards fully electric vehicles. However, mild hybrid systems are not enough to meet carbon emission regulations in several countries, and more advanced technologies are required to avoid fines for the OEMs.
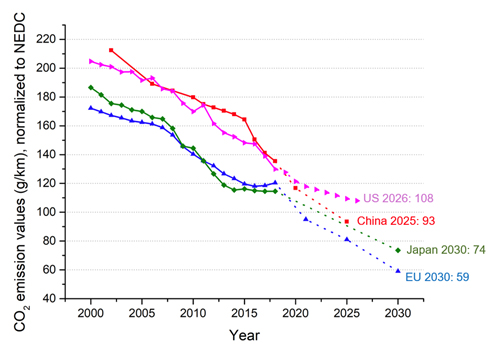
Figure 1: GHG regulation per country / region
As this GHG trend is accelerating, expectations are that the worldwide xEV market might be heading into a time of lengthy expansion, with battery costs also coming down. Tighter restrictions (fuel economy standards/BEV sales regulations) in countries across the board will create demand through around 2025, followed by a transition to a pattern of independent growth from 2025 onward as the core technology gradually becomes cheaper, including batteries.
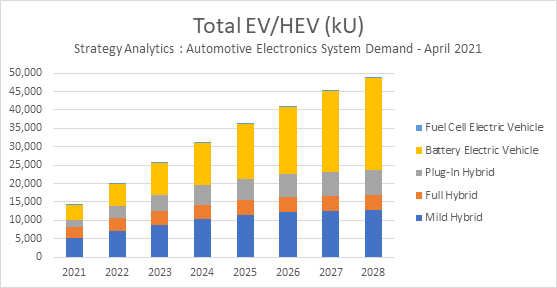
Figure 2: Strategy Analytics - Automotive Electronics System Demand - April 2021 - Global xEV demand forecast of light-duty vehicles (excludes 2/3-wheelers, medium/heavy duty buses & trucks etc.)
HEV system requirements and concept
PHEV and FHEV especially, depending on the respective system concepts, have a higher complexity from a cooperative control strategy point of view (ICE and Electric drive), and on top of that, they are more sensitive to space restrictions of the application components simply because of the combination/addition of the ICE and e-drive functions. This is not only true for the electro-mechanical components but for the electronics as well, such as the digital chipset, analog and power components.
The described system complexity results from the following top-level functionality: At vehicle deceleration, kinetic energy is converted into electric energy by the e-motor and stored in the battery. During acceleration, electric energy from the battery is used to assist the ICE, thus reducing fuel consumption. Having a high-powered e-motor for an FHEV means having a high generator capacity so that more kinetic energy can be recovered (or recuperated) during deceleration, thus resulting in an improvement of fuel efficiency in the range of several 10s of percent.
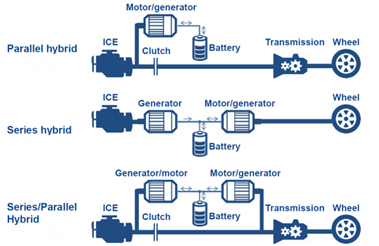
Figure 3: HEV top-level, Global Market Watch #504[Market and technology trends of xEV]
Before introducing a silicon IP concept that will help to solve the requirements complexity and space constraints mentioned above, let us briefly outline the challenges in HEV control. There are several kinds of hybrid architectures, where Figure 3 describes a top-level overview.
The simplest one is a Parallel hybrid system. A motor is placed in parallel with an ICE. The motor/generator assists acceleration by using electric energy from the battery and recharges the battery by using the motor as generator during deceleration. The benefit of this system is lower cost and less control complexity.
In the case of the Series Hybrid system, the kinetic energy created by the ICE is converted to electrical energy by a generator, and then the electrical energy is used to generate kinetic energy again by another motor. This may seem like it is wasting cost and energy. However, the advantage of this approach is that it allows operating the ICE in the most fuel-efficient speed/torque range. An ICE has poor fuel efficiency at low speeds (e.g., < 1500rpm), high speeds (e.g., > 4000rpm), and low torque regions.
The Series/Parallel hybrid is the most complicated system. When the ICE is operating in a fuel-efficient speed/torque range, the ICE output can be directly transferred to the wheels through a clutch and transmission. If torque assistance is needed, the e-motor can assist with acceleration and the ICE can save fuel like in a Parallel hybrid system. When vehicle speed is very slow, the clutch is disengaged, and the system behaves as a Series hybrid to avoid operating the ICE in a poor fuel-efficiency range.
In the case of Series and Series/Parallel hybrid system configurations, usually a combination of two motor/generator devices needs to be tightly and inter-dependently controlled.
HEV control: key challenge and solution
From the previously introduced traction motor system concepts, it becomes obvious that the respective control and synchronization efforts, especially in the case of the Series/Parallel hybrid system are complex because of a high communication load between both entities as well as increased diagnostic efforts to maintain the targeted ASIL-level.
An obvious solution to optimize these efforts is to integrate both Inverter control systems into a one ECU, operated by a single and highly Microcontroller (MCU). By using such a concept, the synchronization between both Inverter control loops can be implemented within one controller, resulting in high communication bandwidth and short latency. Furthermore, the diagnostics & Functional Safety concepts will become more straightforward with the selection of an ASIL-compliant target device. Another benefit of an integrated solution is certainly a strongly optimized Bill-of-Material (BOM), which goes along with reduced component space requirements, both highly welcome effects for the overall system concept.
Solution: MCU with integrated xEV support features
A key asset for HEV application specific MCUs is to offload the calculation process of vector math for the motor control algorithm to a dedicated processing IP. By using this method, the MCU can be equipped with a smaller number of CPU cores while taking over other software tasks, as described above.
Enhanced motor control unit (EMU3)
The embedded 'Enhanced Motor control Unit 3' (EMU Gen3) is a set of individual motor control accelerator modules that calculate the 3-phase PWM compare values using a vector control algorithm, generating rectangle wave patterns based on the motor current values measured by an A/D converter. Additionally, the motor's angle value is obtained through an integrated 'Resolver to Digital Converter' (RDC3A), performing the position sensor interface function. The calculation results of the EMU3 are used by the TSG3, a 3-phase motor timer, to output PWM and rectangle waves.
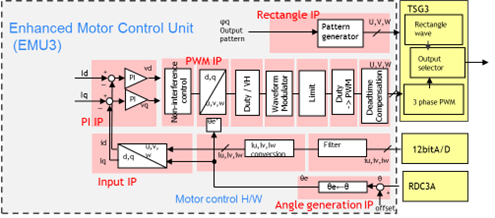
Figure 4: Enhanced Motor control unit
Due to the HW-acceleration of vector math operations the EMU3 IP can calculate the next PWM setting value in a very fast manner.
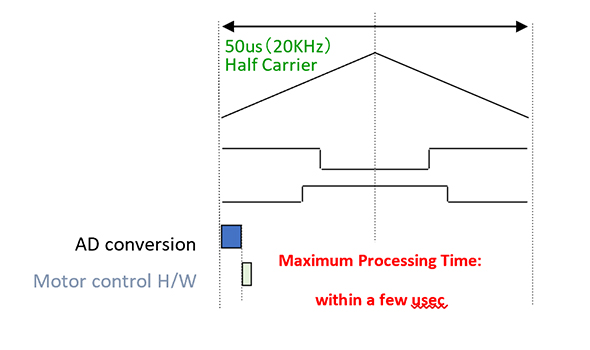
Figure 5: EMU3 performance
The EMU3 IP can exercise motor-control functions in combination with user specific software interventions between any of its functional blocks. Thus, a flexible control concept combining hardware acceleration and individual user software can be realized.
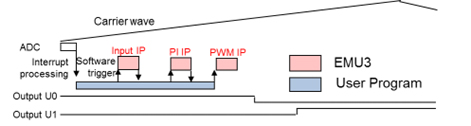
Figure 6: Flexible motor-control in combination with user specific software interventions
Dual e-motor/generator control
The key solution to achieve dual e-motor/generator control capability is based on how the previously introduced motor-control IP ('EMU3'), as well as the embedded position sensor interface are incorporated into the Microcontroller system.
The following diagram shows the actual approach to control two e-motors (please refer to the Appendix for abbreviation definitions):
- CPU2 and CPU3 each controlling one motor respectively. By using the EMU3, the processing of performance-intensive motor-control algorithms, like the Park/Clark-transformation for PWM pattern generation, is shifted from the CPU to the EMU3. This allows the movement of other important software tasks, like diagnostic processing, to be performed by the CPUs.
- CPU1 can be used for other functions: i.e., to realize DC/DC converter control as an optionally integrated add-on feature to optimize the overall HEV system layout. Other functions comprise communication control and “housekeeping functions”, including diagnostics.
- RDC3A is the MCU integrated (Tamagawa AU6805-equivalent) dual Resolver-to-Digital Converter interface, or more generically, the motor position sensor interface, capable of connection to analog resolver- or inductive position sensor signals.
- Concerning position sensing, several OEMs are starting to follow a trend to replace conventional resolver concepts by much more cost-efficient inductive position sensor solutions. Here, the physical principle of Eddy currents supports to detect the position of a simple metallic target moving above a set of coils printed on a simple PCB. Renesas’ inductive position sensing technology, the magnet-free IPS2550 delivers speeds up to 600krpm (electrical) and is designed around the motor, accommodating both off-axis (through shaft and side shaft) and on-axis positioning.
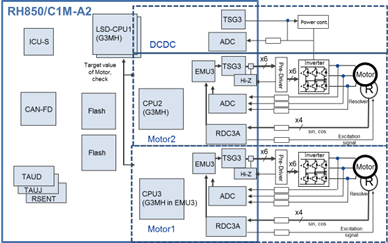
Figure 7: System example to control dual e-motor/generatorSummary
Hybrid vehicles that operate based on e-drive / ICE combinations are especially in need of cost-efficient and size-optimized propulsion systems due to the increased system complexity. Traction motor specific high-performance microcontrollers (MCUs) included with a dedicated HW accelerator function for vector math calculations can help to achieve an overall optimized electronic and electro-mechanic system design.
Solution offering by Renesas
At Renesas, generations of well-proven concepts for HEV-control exist within the 40nm Microcontroller, the RH850/C1M-Ax (R7F701275EABG). This device and its soon-to-be released 28nm successors focus on Inverter control functions for traction motors. As a comprehensive system solution in line with the MCU, Renesas is offering a suitable PMIC (RAA270000KFT), an inductive position sensor (IPS2550DE1R), gate driver (R2A25110KSP) and IGBT devices (IPS2550DE1R). On top, Renesas’ reference SW (Application Note base) as inverter turnkey solution can significantly reduce customers’ R&D efforts (see Fig. 8).
The ASIL-C compliant RH850/C1M-Ax is equipped with an RH850 32-bit G3MH lock-stepped CPU core, operating at a frequency of 240MHz for the C1M-A1. There are two G3MH cores, one of which is lock-stepped, operating at a frequency of 320MHz in the C1M-A2. Along with ROM, RAM and DMA, these devices include various timers such as a motor control timer (TSG3), various serial interfaces including CAN (CAN FD compatible), a 12-bit A/D converter (ADC), an R/D converter (RDC3A) that converts the resolver output signal to digital motor angle data, and a sub-CPU and parallel motor control unit (EMU3), providing peripheral functions that are ideal for motor control in HEVs & EVs. A comprehensive Eco-system with tools incl. model-based development is completing this comprehensive traction motor control solution.
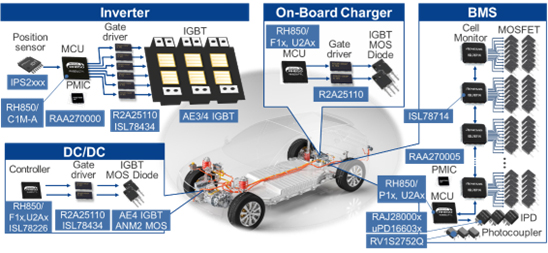
Figure 8: Renesas xEV portfolio
Abbreviation | Description | Background |
---|---|---|
xEV | Comprising BEV, FHEV, PHEV, MHEV | Umbrella term |
PHEV | Plug-In HEV | HV battery with >;40KW e-motor |
FHEV | Full (strong) HEV | HV battery with 20-40KW e-motor |
MHEV | Mild HEV | 48V battery with 5-13KW e-motor |
ICE | Integrated Combustion Engine | Conventional combustion engine |
MCU | Microcontroller Unit | |
EMU | Enhanced Motor control Unit | |
RDC | Resolver to Digital Converter | |
TSG3 | A 3-phase motor timer | |
PWM | Pulse Width Modulation | |
A/D or ADC | Analog to Digital Converter | |
ICU-S | Integrated Cryptography Unit | |
CAN (FD) | Controller area network – Flexible data rate | |
TAUx | Timer Units | |
RSENT | SENT interface | |
SEooC | System element out of context |